Immune microenvironment and immunotherapy in pancreatic cancer: a narrative review
Introduction
Pancreatic cancer is a malignancy of the digestive system, whose incidence is on the rise, while the prognosis of it is almost the worst of all types of solid tumors (1). In the United States, for example, pancreatic cancer is the third leading cause of cancer death in the country, and among all kinds of solid tumors, it has the lowest 5-year survival rate, with about 11% (2). Global cancer statistics in 2020 show that pancreatic cancer is the seventh leading cause of cancer death in both sexes, and the median survival of pancreatic cancer patients is less than 12 months (3). Although a variety of treatments, including surgery, radiation therapy, chemotherapy, and immunotherapy, have been applied to the treatment of pancreatic cancer, the effectiveness is still very limited, and the prognosis is still very poor. Surgical resection combined with adjuvant therapy is the only sensible option at present (4,5). However, due to the occlusion of pancreatic cancer, the difficulty of early detection, and its rapid progression, most patients have no chance for surgery when the diagnosis is confirmed (6). Because traditional therapies struggle to provide patients with better outcomes, many emerging options, including immunotherapy, are receiving increasing attention. Many immunotherapies have achieved gratifying results in the experimental phase, but in clinical applications, immunotherapy has not yet provided significant benefits to patients, and some side effects have also been reported (7). With the deepening of related research, the immunosuppressive microenvironment of pancreatic cancer has gradually been discovered, which is mostly why immunotherapy has little effect (8). Many scholars have conducted detailed studies on the composition of the immunosuppressive microenvironment of pancreatic cancer and its role in tumor progression. Their findings will set the foundation for future advances in immunotherapy. There has been much literature reviewing the tumor microenvironment (TME) of pancreatic cancer as a whole. However, few reviews that explain the relationship between the microenvironment and pancreatic cancer treatment from an immunological perspective. This paper not only describes the components of the microenvironment of pancreatic cancer and their mechanism of action from an immunological point of view but also describes the principle and progress of related immunotherapy, and a future perspective was elaborated in the end. Our goal is to enable researchers to understand the immune microenvironment of pancreatic cancer better, generate more ideas, and ultimately make more remarkable progress through this review. We present the following article in accordance with the Narrative Review reporting checklist (available at https://pcm.amegroups.com/article/view/10.21037/pcm-22-55/rc).
Methods
This is a narrative review. The PubMed database was searched using the keywords “Immune microenvironment”, “Pancreatic cancer”, “Tumor-associated macrophages”, “MDSCs”, “Tregs”, “Tregs and pancreatic cancer”, “Tregs and TME”, “Pancreatic stellate cells”, “Cancer-associated fibroblasts”, “PSCs and pancreatic cancer”, “and pancreatic cancer”, “Immunotherapy”, “Immune checkpoint inhibitors”, “PD-1/PD-L1”, “CTLA-4”, “Oncolytic virus”, “Tumor vaccines”, “Monoclonal antibody”, “Monoclonal antibody and pancreatic cancer”. The date of the literature was limited from January 1st, 1990 to July 1st, 2022. We reviewed and summarized the current literature about the immune microenvironment of pancreatic cancer and related immunotherapies (Table 1). We excluded articles not published in English.
Table 1
Items | Specification |
---|---|
Date of search | August 22nd, 2022/September 1st, 2022 |
Databases and other sources searched | PubMed |
Search terms used | “Immune microenvironment” [MeSH] |
“Pancreatic cancer” [MeSH] | |
“Tumor-associated macrophages” [MeSH] | |
“MDSCs” [MeSH] | |
“Tregs” [MeSH] | |
(“Tregs” [MeSH]) AND “pancreatic cancer” [MeSH] | |
(“Tregs” [MeSH]) AND “TME” [MeSH] | |
“Pancreatic stellate cells” [MeSH] | |
“Cancer-associated fibroblasts” [MeSH] | |
(“PSCs” [MeSH]) AND “pancreatic cancer” [MeSH] | |
(“CAFs” [MeSH]) AND “pancreatic cancer” [MeSH] | |
“Immunotherapy” [MeSH] | |
“Immune checkpoint inhibitors” [MeSH] | |
“PD-1/PD-L1” [MeSH] | |
“CTLA-4” [MeSH] | |
“Oncolytic virus” [MeSH] | |
“Tumor vaccines” [MeSH] | |
“Monoclonal antibody” [MeSH] | |
(“Monoclonal antibody” [MeSH]) AND “pancreatic cancer” [MeSH] | |
Timeframe | January 1st, 1990 to July 1st, 2022 |
Inclusion and exclusion criteria | Excluded non-English publications |
Selection process (who conducted the selection, whether it was conducted independently, how consensus was obtained, etc.) | Xiaoning Yu independently reviewed and selected studies from PubMed |
MDSCs, myeloid-derived suppressor cells; Tregs, regulatory T-cells; TME, tumor microenvironment; PSCs, pancreatic stellate cells; CAFs, cancer-associated fibroblasts; PD-1, programmed cell death 1; PD-L1, programmed cell death 1 ligand 1; CTLA-4, cytotoxic T-lymphocyte associated protein 4.
Immune microenvironment of pancreatic cancer
As elaborated above, the immune microenvironment of pancreatic cancer is gradually entering the field of scholars. The specific immune microenvironment is composed of immune cells, including myeloid-derived suppressor cells (MDSCs), tumor-associated macrophages (TAMs), dendritic cells (DCs), regulatory T-cells (Tregs), and cell matrix components related to tumor immunity, including pancreatic stellate cells (PSCs), cancer-associated fibroblasts (CAFs), extracellular matrix (ECM), I will explain their role in the development of pancreatic cancer below.
Immune cells
MDSCs
MDSCs are a group of cells derived from bone marrow hematopoietic stem cells (HSCs). In pathological conditions such as chronic inflammation and cancer, HSCs perform a large amount of bone marrow hematopoiesis (9). The same pathological conditions can lead to upregulation of the expression of multiple inflammatory factors in the body. Under the stimulation of inflammation-related signals, immature myeloid cells do not differentiate into monocytes and granulocytes as usual but deviate from the original differentiation path and differentiate into a group of cells with immature phenotype and morphology and weak phagocytic function. This is what we currently call MDSCs (10-12). As early as 2011, Condamine proposed a 2-phase model to describe the proliferation of MDSCs (13). Subsequently, in 2020, Karin supplemented and revised this model, proposing a 4-phase model (steps 1–4: myelopoiesis, mobilization in the blood, homing to the tumor site, retention at the tumor site), which is close to a perfect one (14). MDSCs are not present in normal pancreatic tissue. In the state of pancreatic intraepithelial neoplasia (PIN), a small number of MDSCs can be found in the tissues surrounding the pancreas lesion and in the spleen. As pancreatic lesions progress, when PIN progress to pancreatic cancer, the number of MDSCs in pancreatic tissues and spleen increases significantly (15), which suggests that MDSCs may not play a significant role in the relatively early stage of tumor immune microenvironment. Some in vitro experiments have found that MDSCs can inhibit specific anti-tumor T cell responses (16-18), and this effect has also been supported to some extent in in vivo experiments (19-21). Regardless of the animals’ age or the degree of tumor burden, there was a negative correlation between the number of MDSCs and CD8+ T cells (15). This negative correlation echoes the immunosuppressive effects of MDSCs.
With the continuous development of studies, multiple mechanisms of the immunosuppressive effects of MDSCs have also been reported. Currently, there are four main immunosuppressive mechanisms of MDSCs: (I) arginase (ARG). Although studies of arginine metabolism in the process of tumorigenesis and development were noted decades ago, attention has increased in just recent years. ARG has two main types, one (ARG1) is present in the cytoplasm, and the other (ARG2) is present in the mitochondria (22). The primary role of ARG is to break down L-arginine into L-ornithine and urea, which affects the tumor from two aspects. On the one hand, L-ornithine, the decomposition product of L-arginine, is an important substrate for the generation of polyamines, which are fully proven to be one of the essential factors in promoting tumor growth (23). On the other hand, L-arginine can promote the proliferation of T cells. Once it is depleted, it will reduce the expression of cyclins D3 and CDK4 in T cells (24), thus inhibiting the proliferation of T cells. (II) Inducible nitric oxide synthase (iNOS). iNOS is a member of the NOS family (25), and has been found to have a high expression level in MDSCs. Many cytokines such as vascular endothelial growth factor (VEGF), granulocyte-macrophage colony stimulating factor (GM-CSF), interleukin (IL)-6, etc., can induce high expression of iNOS in the bone marrow (26-29). The main function of iNOS is to produce NO, thus inhibiting the function of T cells in many ways. On the one hand, when T cells in the mitotic phase are exposed to a relatively high concentration of NO, the JAK3 and STAT5 pathways within the cells are activated, which causes T cells to be stopped in the G0/G1 phase of the mitosis (30,31). On the other hand, NO can inhibit the body’s immunity against tumors by inhibiting the expression of major histocompatibility complex (MHC) II molecules (32) and promoting T cell apoptosis (33). (III) Reactive oxygen species (ROS). The production of ROS is also considered an important mechanism for MDSCs to suppress the immune response. Various substances, including tumor growth factor β (TGF-β) and IL-6, can induce MDSCs to produce ROS (34). Studies have reported that under in vitro experimental conditions, selective inhibition of ROS production in MDSCs isolated from tumor mice will lead to the disappearance of the immunosuppressive effect of MDSCs (35,36). (IV) Peroxynitrite. It has been found that high expression of peroxynitrite is a crucial feature of MDSCs in the immune microenvironment. This feature strongly correlates with the high expression of ARG and iNOS in MDSCs (37). The peroxynitrite on the surface of MDSCs directly contacts the surface of the T cells, causing the T cell receptor (TCR) and CD8 molecules to react with the nitro group. This process that hinders their binding to the antigen peptide, which subsequently leads to the result that the T cells lose their response to specific tumor antigens (38,39). In addition to the above four mechanisms, MDSCs secrete IL-10 under the influence of interferon γ (IFN-γ) to inhibit T cell function (40,41), and changes in the expression of substances such as programmed cell death 1 ligand 1 (PD-L1), L-selectin (42) are all mechanisms of the immunosuppressive effect of MDSCs.
TAMs
TAMs are important immune cells in tumor tissue, and these cells play a vital role in the genesis, development, metastasis, and immune escape of pancreatic cancer (43). The sources of TAMs are not the same as most macrophages in the common tissue. Traditionally, most macrophages come from yolk cyst cells, while TAMs are derived from monocytes in the bone marrow (44). Under the action of complex cytokine networks, TAMs can mainly differentiate into classically activated M1 macrophages and alternatively activated M2 macrophages (45,46). The process of macrophage differentiation is often referred to as polarization. In the traditional view, M1 and M2 macrophages are often seen as two separate populations, but some studies have found that they may transform into each other under certain conditions (47,48). In the immune microenvironment of tumors, M1 macrophages produce substances such as tumor necrosis factor α (TNF-α), IL-12, IL-23, reactive nitrogen intermediate (RNI), etc., which are manifested as promoting inflammation and anti-tumor effects (49,50). In contrast, M2 macrophages, activated by glucocorticoids, IL-4, and IL-13, secrete IL-10 and TGF-β, which promote the proliferation of Th2 cells, thereby exhibiting inhibition of inflammation and pro-tumor effects (49-52). Existing studies have shown that M2 are much more numerous and valuable in pancreatic ductal adenocarcinoma (PDAC) than M1 macrophages (53). Moreover, the number of M2 macrophages was negatively correlated with the prognosis and survival of patients and positively correlated with the metastasis and immune evasion of tumors (54). At present, there are many studies on TAMs, and their role in pancreatic cancer is gradually becoming clear (55-57). First, TAMs play an important role in pancreatic cancer. In the development of pancreatic cancer, some acinar cells with high plasticity in normal pancreatic tissue, which are stimulated by some inflammation will have the characteristics of ductal cells. This process is called acinar duct metaplasia (ADM). If this adverse stimulation persists, ADM will progress to PIN, which further progresses to PDAC. In the process of pancreatic lesion progression, mutations of the KRAS gene can significantly increase the rate of progression of lesions (53). Since TAMs are the earliest cells that play an immune role in pancreatic inflammation (58) compared to MDSCs (15), their infiltration can amplify inflammatory stimuli. Not only that, TAMs mainly consist of M2 macrophages that can promote mutations in the KRAS gene of pancreatic cells, and the mutated KRAS gene can also promote more macrophages to polarize to M2 (59). This creates a positive-feedback pathway that accelerates the progression of the tumor. IL-6 and IL-10 secreted by TAMs can activate the STAT3 cell pathway in pancreatic cells, improving their sensitivity to inflammatory stimuli (47). Recent studies have also shown that some growth factors, C-C motif chemokine ligand (CCL)5 and TNF-α, etc., can synergistically activate the necrosis factor κB (NF-κB) and phosphatidylinositol 3-kinase (PI3K) pathways within M2 cells and other cells to induce ADM (60,61). TAMS can also promote the combination of heparin and endothelial growth factor receptor (EGFR), which also plays a role in inducing ADM (47) (Figure 1). Second, TAMs are involved in constructing the immunosuppressive microenvironment of pancreatic cancer and helping tumors spread and immune escape. TAMs in the PDAC immune microenvironment can secrete substances such as IL-10, TGF-β, CCL17, CCL12, prostaglandin E2 (PGE2), ARG1, etc., which can downregulate CD8+ T cell activity (62-64). TAMs can upregulate signaling of immune checkpoints at tumor cell, including increased expression of cytotoxic T-lymphocyte associated protein 4 (CTLA-4) and PD-L1, blocking T cell immune recognition and losing response to tumor cells (64,65). TAMs are also involved in the invasion and progression of tumors, and an important mechanism is the bilateral promotion of VEGF and TAMs. Studies have shown that TAMs can be enriched in the hypoxic and no-vascular areas of tumors and upregulate the expression of VEGF in this region (66). VEGF can promote blood vessel formation and facilitate tumor spread. Further studies confirmed that VEGF could also recruit TAMs to infiltrate tumor tissue (67). The formation of this positive feedback pathway is exceptionally conducive to tumor invasion and spread and provides a possible theoretical basis for early hematogenous metastasis.
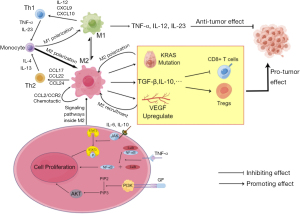
Tregs
In the TME of pancreatic cancer, infiltration of Tregs is an important feature. Since Tregs’ primary function is to regulate immune system function, its role in the tumor immune microenvironment is also considered to negatively regulate immune function (68). Several studies have confirmed Tregs’ role in tumors’ immunosuppressive microenvironment. For example, the increase of Tregs in cancer tissue and peripheral blood is directly related to poor prognosis (69). Some treatments targeting at Tregs which aim to weaken Tregs’ function have also been proven effective (70,71). Tregs are habitually divided into two groups. One is the natural Tregs (nTreg), which persistently express forkhead box P3 (FOXP3) at a high level and play an immunomodulatory role through direct contact with other cells (72). Another group is called induced Tregs (iTreg). They can be activated by T cell antigen recognition receptors and secrete cytokines includes mainly TGF-β, which act as an immunosuppressor. However, some scholars prefer the new naming method according to Tregs’ origin (73). Tregs derived from the thymus gland are called tTreg, while those from the periphery are called pTreg. This nomenclature has certain benefits, but its main problem is the lack of specific markers to distinguish them. At present, in addition to NR1 and Helios, which have been studied to a certain extent (74-76), other specific markers that can be used to distinguish the two are still limited. Tregs can also be divided into different phenotypes according to the expression of substances such as CTLA-4, FOXP3, CD69, programmed cell death 1 (PD-1), etc. For example, Yadav divided Tregs into the following three types: activated Treg for CD45RA−FOXP3+, resting Treg for CD45RA+FOXP3−, and non-Treg for CD45RA−FOXP3− (77).
There are many studies on Treg’s involvement in the immune microenvironment, but the following two conclusions are clear. (I) Tregs participate in the immune microenvironment by secreting immunosuppressive cytokines. TGF-β and IL-10 are representatives (78,79). These cytokines can act directly on natural killer T (NKT) cells, reducing their activity (80). They can also act on DCs (81,82), then affect the proliferation of cytotoxic T lymphocyte (CTL) and effector T cells. (II) Tregs participate in the process of tumor immunity by upregulating the expression of immune checkpoint molecules such as CTLA-1 and PD-1/PD-L1. Upregulation of CTLA-4 expression is an important mechanism for Treg’s involvement in immune microenvironment. In normal tissues, once a potent combination of MHC and TCR occurs, the expression of CTLA-4 rises, inhibiting T cell activation, which is a potential protective mechanism to protect the body from severe immune damage (83). During the activation of normal T cells, the combination of CD28 and CD80/86, which provides a co-stimulating signal, is essential. CTLA-4 is homologous with CD28, it can also bind to CD80/86, but this kind of binding cannot provide a co-stimulation signal, however, it will hinder the binding of CD28 and CD80/86 through the mechanism of competition and then lead to the obstruction of T cell activation (84,85). The role of PD-1/PD-L1 is similar to that of CTLA-4. If the binding of MHC to TCR and the binding of PD-1 to PD-L1 occur simultaneously on the surface of T cells, the activity of such T cells will be inhibited, and the secretion of TNF-α and IFN-γ will also be reduced (86,87). Nevertheless PD-1/PD-L1 also differs from CTLA-4. CTLA-4 is mainly expressed intracellularly and will be transferred to the cell surface when T cells are stimulated (83). Therefore, the CTLA-4-mediated immunosuppressive response mainly occurs before the effect phase. While PD-1/PD-L1 is on the surface of activated T cells, so the immunosuppressive response it mediates occurs during the effector phase (88).
Of course, Tregs can interact with many components in the immune microenvironment, and the complete action network is still undiscovered. For example, it is reported that ectonucleotidases and CD39/73, a molecule that is rare in normal people but can be detected in patients with head and neck tumors (89), synergistically use exogenous adenosine triphosphate (ATP) to produce immunosuppressive adenosine, which may have a relation with the suppressive activity of Tregs (90). Shockingly, some new studies have found that Treg cells may have somehow inhibited tumorigenesis (75,91,92), which is more pronounced in some malignant tumors of the airway and digestive tract. Because such organs and tissues are often exposed to a mixture of air, secretions, and microbe for a long time, such an environment is often more likely to lead to inflammation, which is the most likely origin of many tumors. It is related to the DNA instability and pro-angiogenesis effect brought by inflammation to cells. Some report notes that the immune tolerance induced by Tregs in inflammation may potentially protect the body from tumor distress (93-96).
Other cells
In the complex immune microenvironment of pancreatic cancer, there are not only the above three immune cells but a variety of cells, including DCs, B cells, monocytes, and neutrophils, are also involved in the microenvironment. Jang’s research noted that DCs in the TME of pancreatic cancer patients could express indoleamine 2,3-dioxygenase (IDO), calming the intense immune response of T cells down (97). γδT cells can inhibit the activation of anti-tumor αβT cells through PD-1/PD-L1 mechanisms that are similar to Tregs (98). The immune microenvironment of pancreatic cancer is a complex whole in which immune cells interact to form a network, and the complex connections have yet to be explored.
Cell matrix components
PSC and CAF
PSCs play a non-negligible role in the progression of pancreatic cancer, which not only promotes pancreatic cancer to infiltrate surrounding areas and metastasize to distant places but also participates in the composition of the immune microenvironment (99) (Figure 2). PSCs can interact with immune cells, secrete cytokines, and convert to CAFs (100). In normal pancreatic tissue, PSCs are inactive, producing many ECM components or degrading them by secreting enzymes (101). Therefore, PSCs can maintain the ECM in homeostasis in normal pancreatic tissue. However, once PSCs are stimulated by inflammation, they are activated, and these PSCs are more inclined to produce ECM components than to degrade them. When PSCs are activated, the lipid droplets full of vitamin A in their cells disappear, and PSCs express a considerable amount of alpha smooth muscle actin (α-SMA) (102). If this inflammatory stimulus is chronic and persistent, then PSCs will be continually affected by activation signals and cannot accept the apoptosis signal, which is why the apoptosis process of PSCs is not occurring. Continuously activated PSCs secrete large amounts of ECM components, which are mainly fibrous components (103). It is thought to be the reason why PDAC tissue is highly fibrous. Other studies have pointed out that PSCs not only accept stimulus signals from other cells or molecules, but once the activation signal appears, PSCs will promote self-activation and proliferation through autocrine. Validated autocrine components include IL-1, IL-6, IL-8, TNF-α, TGF-β, etc. (104,105). In addition, the relationship between PSCs and some immune cells has been elucidated. Studies have shown that PSCs can prevent CD8+ T cells from migrating to tumor sites (106), which may be related to the contact effect of the fiber components with T cells. It has also been shown that PSCs can recruit MDSCs to infiltrate the tumor site and can, enhance the activity of MDSCs, and promote their differentiation (107). Some studies point out that PSCs play a positive role in helping MDSCs infiltrate the tumor site, and increase in the activity of MDSCs and the acceleration of MDSCs differentiation have also been confirmed to be related to PSCs (107-109).
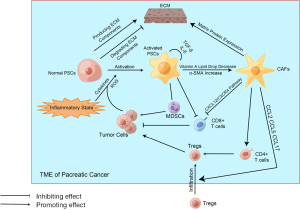
CAFs also influence the immune microenvironment of pancreatic cancer (Figure 2). Currently known studies have identified its association with the fibrotic matrix component of pancreatic cancer and its resistance to chemotherapy drugs, including gemcitabine (110). CAFs are a class of cells that are not proliferative and do not belong to the category of endothelial cells or immune cells but can produce ECM components (111). In pancreatic cancer’s TME, the main source of CAFs is activated PSCs. Although CAFs are matrix components, they can still be involved in forming inhibitory immune microenvironments in various ways. First, CAFs can affect the chemotaxis of effector T cells through the CXCL12/CXCR4 axis, preventing them from infiltrating the tumor site (112). Second, CAFs can also mediate monocytes’ and Tregs’ infiltration by secreting chemokines including CCL5 (112). Moreover, monocytes are an essential source of MDSCs. Some CAFs can express MHC-II molecules but cannot express co-stimulating molecules, leading to the transformation from CD4+ T cells to Tregs (113). There is much more research on CAFs-mediated immunosuppressive microenvironments, and treatments that target CAFs have had some effect in animal models, which may be related to the increased activity of CTL after CAFs reduction (114).
Other components
Among the cellular matrix components of pancreatic cancer, there are many components that can mediate the formation of inhibitory immune microenvironments to varying degrees through many different mechanisms. For example, stromal proteins can physically block the contact between anti-tumor immune cells and tumor cells (115); hyaluronic acid can bind water molecules in large quantities to construct an environment of high fluid pressure which act as a barrier from substances such as chemotherapy drugs elsewhere (116). The epithelial to mesenchymal transformation (EMT) process, which is highly representative in pancreatic cancer TME, can also promote tumor proliferation and metastasis (117). In general, the cellular matrix composition of pancreatic cancer TME is very complex. It is still a long way from fully understanding it and applying its principles to clinical practice.
Progress in immunotherapy for pancreatic cancer
As mentioned earlier, traditional therapies still do not provide satisfactory results for patients, especially for metastatic lesions, and we lack effective treatments. This is the background to the rise of immunotherapy. Therapies like tumor vaccines, chimeric antigen receptor T-cell (CAR-T) immunotherapy, immune checkpoint inhibitors, monoclonal antibody therapies, oncolytic viruses, and therapies targeting the stromal components of TME cells are being increasingly studied, and these immunotherapies are also seen as potential means of conquering pancreatic cancer. In animal experiments, many immunotherapies have achieved gratifying results, and some drugs have entered phase II clinical trials. However, immunotherapy also has some problems, the most important of which are severe autoimmune responses and even patient death. Despite all this, it is believed that shortly, immunotherapy will receive more attention, the problem will be solved, and more drugs will be put into clinical application, which will undoubtedly benefit more patients.
Tumor vaccines
Tumor vaccines are an emerging therapy among the currently known immunotherapies, and its effects have been initially affirmed. We can use DNA or peptides as antigens to activate immune cells, traditionally known as the vaccine principle. We can also directly utilize activated DCs or complete tumor cells as components of vaccines. An ideal vaccine target should be a class of substances expressed only in tumor cells and not in normal cells. Unfortunately, no such proteins have been found in pancreatic cancer tissues, so it is a more reasonable choice to develop a vaccine by taking advantage of differences in the expression levels of certain substances in tumor cells and normal cells. Research on carcinoembryonic antigen (CEA), human epidermal growth factor receptor 2 (HER2), mucin 1 (MUC1), P53, etc., as vaccine targets has been reported (118-121). Traditional vaccines tend to use autologous cells treated with radiation. However, in the treatment of pancreatic cancer, not many patients have the chance to undergo surgery, and even if a small number of people are able to do so, they will eventually be unable to gain enough autologous tumor cells for various reasons (122). Therefore, using allogeneic tumor cells in vitro culture as a stable source of tumor cells has become the primary method of preparing pancreatic cancer tumor vaccines (123). Today, the pancreatic cancer tumor vaccine that has been fully studied is mainly the GVAX vaccine. It uses genetic engineering methods to reconstruct pancreatic cancer cells and make them highly express GM-CSF, thus activating the immune system. There is a study showing that GM-CSF rises at the tumor site a few days after GVAX vaccination, which increases the body’s immune response (124). A phase-1 clinical trial showed that 3 of the 8 patients receiving two doses of the GVAX vaccine achieved a disease-free survival effect of 15 years (125). In another phase-2 clinical trial, patients receiving the GVAX vaccine also showed promising clinical efficacy (126). However, some phase-2 clinical trials show that GVAX combined with ipilimumab did not provide survival benefits for patients with pancreatic cancer compared with combination chemotherapy (127). These contradictory results may be related to how the GVAX vaccine is used, including dosage, frequency, and other therapies in combination.
Another emerging vaccine is the Algenpantucel-L vaccine. It consists of two different radiation-treated human pancreatic cancer cell lines that mainly express α-1,3-galactosyltransferase, which can catalyze the formation of α-galactosylated epitopes on cell membranes. A phase-2 clinical trial showed an 81 percent improvement in 1-year disease-free survival for patients using the vaccine and a 96 percent improvement in 1-year overall survival (128). Another phase-2 clinical trial showed that this vaccine is more effective in combination with neoadjuvant chemotherapy (129). In addition, tumor vaccines based on telomerase, mutated KRAS gene, and heat shock protein (HSP) peptide complexes are being studied.
Immune checkpoint inhibitors
Immune checkpoints are defined as programmed death receptors and their ligands. Tumor cells inhibit immune cell activity by expressing immune checkpoint molecules, thereby influencing the host’s immune response to the tumor. At present, the immune checkpoints that have been fully studied are mainly CTLA-4 and PD-1/PD-L1. The antitumor effects of CTLA-4 inhibitors can be traced back to 1996 when a study reported that CTLA-4 antibodies could cause mice to exhibit rejection of transplanted tumors (130). Subsequently, several clinical trials have been carried out (131-133). Among those, one phase-3 clinical trial demonstrated that using ipilimumab, a CTLA-4 antibody, is beneficial for patients with melanoma (134). Although many scholars have noted the anti-tumor effects of CTLA-4 inhibitors, and there are many related drugs in development, the use of CTLA-4 still has some problems and controversies. Studies have shown that the using ipilimumab and nivolumab can lead to a probability of adverse therapeutic reaction, mainly autoimmune diseases. If the two are combined, this probability rises to 60% (135). However, some scholars believe that we can avoid similar adverse reactions by improving drugs. In other words, severe adverse reactions may not be due to their inhibition of CTLA-4. The study reported that among the many CTLA-4 antibodies, the drug that was the most effective produced the least number of antibodies targeting DNA (136). Another study confirmed this from a pathological point of view (137). Du and his colleagues point out that CTLA-4 inhibitors may not appear to be a sufficient condition for tumor treatment, which is subversive in the traditional view of CTLA-4. It was found that CTLA-4 inhibitors did not block that many CTLA-4 and CD80/86 bindings at the cell surface, and even though CTLA-4 antibodies successfully blocked the binding of the two, CTLA-4-mediated physiological responses were observed, while the body’s immunity to tumors was enhanced (138). This finding may suggest the existence of another mechanism related to tumor immunity related to CTLA-4. CTLA-4 inhibitors have a more limited range of use than PD-1/PD-L1 inhibitors. However, scholars are still keen to study the antitumor effects of CTLA-4 inhibitors because many studies have shown that the use of CTLA-4 inhibitors can improve the efficacy of PD-1 inhibitors (139-141).
PD-1/PD-L1 is another target of the immune checkpoint inhibitors. In treating non-small cell lung cancer (NSCLC), U.S. Food and Drug Administration (FDA) has recommended anti-PD-L1 therapy for patients with immunohistochemical PD-L1 positive (142,143). In the treatment of colorectal cancer, the treatment of PD-L1 antibodies combined with PD-L1 secretion inhibitors also yielded comparable results (144). At present, the combination therapy of PD-1/PD-L1 inhibitors with other anti-tumor drugs has entered the field of scholars, which mainly includes the combination of PD-1/PD-L1 inhibitors with CTLA-4 inhibitors, chemotherapy drugs, and targeted drugs. A phase-3 clinical trial of a combination of PD-1 and CTLA-4 antibodies showed significant benefits in patients with melanoma (145), but this also meant more substantial side effects (146). In patients with NSCLC, regimens of PD-1 antibodies in combination with platinum-based chemotherapy are better than those of single agents (147). Several trials in patients with renal cell cancer have also shown that PD-1 antibodies combined with axitinib, a VEGF receptor (VEGFR) inhibitor, and other chemotherapy drugs are more effective than monotherapy (148,149).
Adoptive immunotherapy (CAR-T therapy)
CAR-T therapy is an immunotherapy for the treatment of tumors. T cells are activated and can specifically identify tumor cells by cell designing and engineering, to achieve the effect of killing tumor cells and treating tumors. CAR-T therapy has shown promising efficacy in some hematologic tumors, and a representative example is its use of it in acute B-lymphoblastic leukemia (150). Regrettably, however, CAR-T therapy has not been able to show satisfactory results in treating solid tumors. One reason for this is that many tumors, including pancreatic cancer, are in an immunosuppressive microenvironment, which limits the function of T cells. Therefore, some scholars are studying the combined effects of CAR-T therapy with TME targeting therapies. A more important reason is that solid tumors lack specific targets for designing and engineering T cells. In pancreatic cancer, markers such as mesothelin (MSLN) and MUC1 tend to be overexpressed, and the elevation is inversely correlated with the prognosis of patients (151), providing us with potential targets. Nevertheless, many targets are tumor-associated antigens (TAA) rather than tumor-specific antigens (TSA) (120), which means they exist in normal cells, and this will lead to the “on-target, off-tumor” effect. The so-called “on-target, off-tumor” effect is that CAR-T cells recognize normal cells as tumor cells and attack them, which leads to a variety of autoimmune reactions, including colitis. It even leads to deaths of patients (152,153). Although CAR-T therapy for solid tumors is still not perfect, it is fortunate that in animal experiments, its efficacy of it for pancreatic cancer has been confirmed (154-156), and clinical trial results have also been reported (157). Among the many targets reported at present, CAR-T therapy for MSLN has achieved the best efficacy, while its side effects, mainly consisting of autoimmune reactions, are relatively few (158). The results are satisfying. All pancreatic cancer cells express MSLN, while normal pancreatic cells hardly express this substance, which provides a theoretical basis for avoiding the “on-target, off-tumor” effect (159). There have been some studies reporting the role of MSLN in many types of solid tumors (160-162). However, to fully reveal its principles for tumorigenesis and progression and put them into clinical practice, we still have a long way to go. Studies have shown that in PDAC, specific T-cell responses against MSLN have been observed (163,164). Zheng’s research suggests that MSLN may regulate the growth and apoptosis of tumor cells through p53-mediated pathways (165). Another study has found that the interaction between MSLN and CA125 may be related to the adhesion of tumor cells (166). Related clinical trials are also underway. A small Phase-1 clinical trial shows that CAR-T therapy to MSLN in patients with recurrent and(or) metastatic pancreatic cancer leads to prolonged survival (167).
Immunotherapy targeting components of pancreatic cancer TME
As described previously, pancreatic cancer has a complex immune microenvironment that plays a crucial role in the progression of pancreatic cancer. Therefore, the treatment aimed at the immune microenvironment of pancreatic cancer has been in people’s field of vision. The immune microenvironment of pancreatic cancer consists of cells and matrix components, and accordingly, the treatment begins in these two aspects. (I) Treatments targeted at immune cells. The main targets are TAMs and MDSCs. Some studies have shown that CD40 activation is key to macrophage transition to an anti-tumor phenotype (168), which provides us with a potential therapeutic basis. In addition, some therapies that target cytokines and chemokines are also progressing. The CCL2/C-C motif chemokine receptor 2 (CCR2) axis is the main driving force for TAM chemotaxis and infiltration into the tumor (169). A phase-1 clinical trial showed that CCR2 inhibitors combined with FOLFIRINOX chemotherapy yielded clinical benefits in at least 50 percent of patients (170). The differentiation of TAMs and MDSCs is modulated by colony stimulating factor (CSF)-1/CSF-1R, while the prospect of CSF-1R blockers has been shown. The effect of CSF-1R blockers in the treatment of tumors in a separate combination with CD40 agonists and immune checkpoint inhibitors has been reported (171,172). Activation of the CXCL2/CXCR2 axis can facilitate MDSCs chemotactic to tumors. Accordingly, trials blocking both CXCR-2 and CSF-1R have been published, which shows that this therapy can achieve anti-tumor purposes by improving immune cell infiltration (173-175). (II) Treatments targeted the cell matrix components. One approach is to deplete the tumor matrix, and a representative drug is the hyaluronidase. A study shows that IDO inhibitors combined with hyaluronidase significantly increased the infiltration of antitumor T cells (176). However, other studies have shown that treatment for matrix depletion may lead to pancreatic cancer being more likely to metastasize, possibly because of the loss of the restrictive effect of the dense matrix around tumor cells (177). Another approach is stromal modulation therapy targeting vitamin D and vitamin D receptors. Patients with vitamin D deficiency have been reported to have a relatively poor prognosis (178). Vitamin D receptors are expressed on the surface of PSCs, meaning that vitamin D-associated metabolism is part of matrix metabolism in the TME. Studies have shown that activation of vitamin D receptors can cause a decrease in fibrosis levels in the TME, leading to an improved response of tumors to various chemotherapy drugs, including gemcitabine (179). In addition to vitamin D and vitamin D receptors, fibroblast activated proteins (FAPs) are also associated with highly fibrotic matrix in pancreatic cancer (180), and therapies targeting FAPs are also being studied (181).
Monoclonal antibody therapy
Monoclonal antibody therapy is well-known immunotherapy which can specifically bind to some cell surface receptors or cytokines, block the conduction of signals, weaken their physiological effects, and finally achieve the effect of inhibiting tumor growth and even eradicating tumors. Currently, cetuximab is a common monoclonal antibody that has a very high affinity with the epidermal growth factor receptor (EGFR) but has no physiological activity of EGFR ligands. It can competitively inhibit the activation of EGFR, thereby blocking intracellular downstream signaling pathways and inhibiting tumor cell proliferation. Some clinical trials about the possibility of using cetuximab in pancreatic cancer have shown results. In a phase-2 clinical trial, the combined use of cetuximab and gemcitabine was confirmed to be possible in patients with advanced pancreatic cancer (182). Another retrospective study confirmed that conventional chemotherapy combined with cetuximab and bevacizumab had better efficacy than chemotherapy alone (183). However, the use of monoclonal antibody therapy in pancreatic cancer is controversial. Clinical trials have also shown that adding cetuximab to gemcitabine and cisplatin does not improve survival in patients (184). Besides cetuximab, a number of monoclonal antibodies have also been put into clinical trials, such as FG-3019, which targets connective tissue growth factor (CTGF), and H2Mab-19, which targets HER2.
Oncolytic virus therapy
Oncolytic virus therapy is emerging immunotherapy that achieves anti-tumor effects by attacking tumor cells with particular viruses. Part of the virus’s anti-tumor effect comes from virus-mediated lysis of tumor cells. However, more importantly, the lysis of tumor cells can expose some tumor antigens that are difficult to identify using traditional methods, thus achieving the effect of activating patients’ anti-tumor immunity. At present, some viruses have been studied as ideal sources of oncolytic viruses, including adenoviruses, measles viruses, and herpes simplex viruses (HSV), etc.. On adenovirus, there is still not so much research. Moreover, very few of them can be put into clinical trials and applications. An adenovirus with a defective E1B-55kD gene can proliferate in cells with deficient in the p53 gene to replicate only in pancreatic cancer cells (185). In phase-1 clinical trials, the virus showed excellent safety, with an elevated immune response in patients proved by increased T cell responses and elevated antibody levels (186). However, the virus did not show good clinical efficacy in subsequent clinical trials. Another reported adenovirus-derived oncolytic virus is adenovirus with two defective genes of E1ACR and E1B19K, whose main role is inhibiting cell autophagy. Studies have pointed out that the combined use of gemcitabine and this kind of virus has shown gratifying results in animal models (187), but reliable clinical research data are still lacking. HSV is also considered to be a possible source of oncolytic viruses. An oncolytic virus named FusOn-H2 can replicate in cells where the Ras signaling pathway is activated, making it possible to kill tumor cells accurately (188). Another oncolytic virus, L1BR1, comes from the HSV-2 virus, while the US3 gene is not activatable. The US3 gene can inhibit cell apoptosis. In a small range study, patients with pancreatic cancer benefited from the combination of chemotherapy and the L1BR1 virus (189). Measles virus is also an ideal resource for an oncolytic virus, which enters cells through the CD46 molecule, a cell surface molecule that many tumor cells, including pancreatic cancer cells, highly express. Currently, two kinds of measles virus with MV-NIS gene and prodrug-convertase gene have shown good effect in animal experiments (190,191), but few reports of clinical trials are published. Some representative oncolytic viruses are listed below (Table 2).
Table 2
Viruses | Modification | Target | Effect | References |
---|---|---|---|---|
Adenovirus | ||||
hTERT-Ad | Insertion of E1A gene controlled by hTERT promoter | Cells with upregulated hTERT | Lyse tumor cells | (192) |
ONYX-015 | Deletion of E1B gene | Cells with dysfunctional p53 gene | Lyse tumor cells | (185,186) |
LOAd703 | Insertion of genes encoding CD40L and 4-1BBL | Cells with unbound E2F gene | Lyse tumor cells | (193) |
VCN-01 | Insertion of genes encoding hyaluronidase | Cells with disrupted Rb pathway | Lyse tumor cells and degrade ECM components | (194,195) |
A strain of adenovirus | Depletion of E1ACR2 and E1B19K | Cells with deregulated pRb-p53 pathways | Tumor-specific cytotoxicity | (187) |
Herpes simplex virus | ||||
FusOn-H2 | Deletion of PK domain | Cells with activated Ras pathway | Lyse tumor cells | (188) |
L1BR1 | Defection of US3 gene | A variety of tumor cells | Promote apoptosis | (189) |
OrienX010 | Insertion of genes encoding GM-CSF | Pancreatic cancer cells | Upregulate the expression of GM-CSF | (196) |
Vaccinia virus | ||||
GLV-1h68 | Mutations of F14.5L, J2R and A56R | Pancreatic cancer cells | Lyse tumor cells | (197) |
A lister strain of vaccinia | Insertion of endostatin-angiostatin fuse gene | Cells with high EGFR expression | Lyse tumor cells, inhibit angiogenesis and overcome endothelial cell anergy | (198) |
MV | ||||
A strain of MV | Insertion of MV-NIS gene | Cells with CD46 receptor | Lyse tumor cells and slow tumor growth | (190) |
Another strain of MV | Addition of prodrug convertase gene | Cells with high expression of PSCA | Lyse tumor cells and promote tumor immune exposure | (191) |
Myxoma virus | ||||
Myxoma virus | Insertion of vMyx-GFP and vMyx-tdTr | Cells with activated Akt pathway | Lyse tumor cells (in vitro) | (199,200) |
hTERT, human telomerase reverse transcriptase; ECM, extracellular matrix; GM-CSF, granulocyte-macrophage colony stimulating factor; EGFR, endothelial growth factor receptor; MV, measles virus; PSCA, prostate stem cell antigen; GFP, green fluorescent protein.
Perspective
At present, although the focus of many studies is on the immune microenvironment and immunotherapy of pancreatic cancer, and related research has also drawn considerable attention and make much progress, we still know very little about the microenvironment of pancreatic cancer, with the unsatisfactory progress and clinical effect of immunotherapy. The immune microenvironment of pancreatic cancer is an extremely complex network, and every tiny impact on any point, such as immune cells, non-immune cells and matrix components, can affect the occurrence, progression and treatment effect of pancreatic cancer. In this complex network, the main influence on the immune microenvironment is immune cells and related cytokines, which interact with one another to form an immunosuppressive microenvironment thus promote the occurrence and development of pancreatic cancer. But the immunotherapy for pancreatic cancer is also in a huge gap between ideal and reality. Some immunotherapies, such as oncolytic virus therapy, CAR-T therapy and immune checkpoint inhibitor therapy, can specifically kill tumor cells, which is theoretically highly possible to stop tumor progression, but many clinical trial results prove that we are naive and ignorant to a certain extent. The treatment of pancreatic cancer will remain a huge challenge for some time. The immune microenvironment of pancreatic cancer and related immunotherapies are still frontier in the field. For solid tumors, especially pancreatic cancer, the therapeutic effect of surgery is very limited. Therefore, fully understanding the immune microenvironment of pancreatic cancer, using patients’ own anti-tumor immune response and various types of immunotherapy principles to specifically kill tumor cells will bring a glimmer of hope to overcome pancreatic tumors. It is hoped that with the development of science and technology, pancreatic cancer will be less and less harmful to humans until we can completely overcome this problem.
Conclusions
Pancreatic cancer is a malignant tumor of the digestive system with a very poor prognosis, a high case fatality rate, and a low 5-year survival rate, and R0 resection combined with chemotherapy as a standardized therapy still cannot achieve satisfactory results currently. With the study of the immune microenvironment of pancreatic cancer, it has been found that the inhibitory immune microenvironment of pancreatic cancer is related to the immune incapacitation of patients and related-drug resistance, and it is also an important reason why pancreatic cancer is so difficult to treat. In this paper, we review research in related fields and systematically describe the various components and their interactions in the immune microenvironment of pancreatic cancer. It has become a wide consensus that the stimulation of pancreatic tissue inflammation to pancreatic acinar cells is one of the important reasons for the occurrence of pancreatic cancer. In fact, from the occurrence of inflammation, the formation process of pancreatic cancer immune microenvironment has begun. The first cells to initiate the immune response are TAMs, which can differentiate in 2 directions, but in the pancreatic cancer TME, most TAMs will differentiate into M2 macrophages that exhibit pro-tumor effects. M2 can participate in the formation of the immune microenvironment by promoting gene mutations in acinar cells and secreting immunosuppressive cytokines. MDSCs respond relatively slowly to tumors. They originate in the bone marrow, circulate through the bloodstream and proliferate in the microenvironment. Such cells can either produce immunosuppressive effects mediated by a variety of substances (including ARG, iNOS, ROS, etc.) or induce T cells to differentiate into Tregs by direct contact with effector T cells, hindering their cytotoxic effect on tumors. Some therapies targeting TAMs and MDSCs are being studied, such as CD40 monoclonal antibodies, CCR2 inhibitors, and CSF-1R blockers. Tregs are also an important component of the immunosuppressive microenvironment. The role of Tregs in secreting inhibitory cytokines has been well understood, and another important immunosuppressive mechanism is the upregulation of immune checkpoint molecular expression, for which immune checkpoint inhibitor therapy has made some breakthroughs. In the immune microenvironment, there are not only immune cells but also cell matrix components, mainly composed of PSCs, CAFs, and ECMs. PSCs are activated by inflammation or tumor stimulation, highly express the ECM component, and convert to CAFs. PSCs and CAFs are the leading causes of high fibrosis of pancreatic cancer tissue, which greatly limits the role of exogenous therapeutic drugs and peripherally derived immune cells. Therapies for matrix components have also been reported, mainly matrix depletion therapy with hyaluronidase as the core and matrix modulation therapy with vitamin D receptor agonists as the core. The efficacy and feasibility of the latter are better than the former. Since surgery combined with chemotherapy is not effective enough in the treatment of pancreatic cancer, immunotherapy is considered a promising treatment. In addition to some of the therapies mentioned above that target the components of the immune microenvironment of pancreatic cancer, there are also breakthroughs in immunotherapy (oncolytic virus therapy, CAR-T therapy, tumor vaccines, and monoclonal antibody therapy), and many clinical trials are underway. The breakthrough of immunotherapy has brought a glimmer of light to pancreatic cancer patients, and it is hoped that in the near future, this light will become a burst of shining sunshine, helping us overcome pancreatic cancer and help more patients regain their health. Although the narrative of this review is nearing its end, and its discussion of the immune microenvironment of pancreatic cancer and related immunotherapies is clear, it is undeniable that this review still has its limitations for various reasons. First, due to the single database used to search the literature (PubMed only), some of the relevant studies and literature have not been included in this review. Second, because the authors focused only on the immune microenvironment of pancreatic cancer rather than the TME of pancreatic cancer as a whole, the literature cited and the conclusions drawn are not so comprehensive. Third, although immunotherapy is the hope of pancreatic cancer treatment, certainly, that traditional therapies cannot be abandoned. The treatment of pancreatic cancer should be a combination of multiple therapies rather than a victory of monotherapy. Perhaps in the future, the progress of immunotherapy for pancreatic cancer will reach unprecedented heights, but there is still a great possibility that other therapies will be retained in clinical practice.
Acknowledgments
The authors thank Drs. Xianjun Yu and Si Shi from Fudan University for their participation in the writing of this article and especially their support in the creation of the iconography.
Funding: This study was jointly supported by the National Natural Science Foundation of China (No. U21A20374), Shanghai Municipal Science and Technology Major Project (No. 21JC1401500), Scientific Innovation Project of Shanghai Education Committee (No. 2019-01-07-00-07-E00057), Clinical Research Plan of Shanghai Hospital Development Center (No. SHDC2020CR1006A), and Xuhui District Artificial Intelligence Medical Hospital Cooperation Project (No. 2021-011).
Footnote
Reporting Checklist: The authors have completed the Narrative Review reporting checklist. Available at https://pcm.amegroups.com/article/view/10.21037/pcm-22-55/rc
Conflicts of Interest: All authors have completed the ICMJE uniform disclosure form (available at https://pcm.amegroups.com/article/view/10.21037/pcm-22-55/coif) and report supports from the National Natural Science Foundation of China (No. U21A20374), Shanghai Municipal Science and Technology Major Project (No. 21JC1401500), Scientific Innovation Project of Shanghai Education Committee (No. 2019-01-07-00-07-E00057), Clinical Research Plan of Shanghai Hospital Development Center (No. SHDC2020CR1006A), and Xuhui District Artificial Intelligence Medical Hospital Cooperation Project (No. 2021-011). The authors have no other conflicts of interest to declare.
Ethical Statement: The authors are accountable for all aspects of the work in ensuring that questions related to the accuracy or integrity of any part of the work are appropriately investigated and resolved.
Open Access Statement: This is an Open Access article distributed in accordance with the Creative Commons Attribution-NonCommercial-NoDerivs 4.0 International License (CC BY-NC-ND 4.0), which permits the non-commercial replication and distribution of the article with the strict proviso that no changes or edits are made and the original work is properly cited (including links to both the formal publication through the relevant DOI and the license). See: https://creativecommons.org/licenses/by-nc-nd/4.0/.
References
- Ilic M, Ilic I. Epidemiology of pancreatic cancer. World J Gastroenterol 2016;22:9694-705. [Crossref] [PubMed]
- Siegel RL, Miller KD, Fuchs HE, et al. Cancer statistics, 2022. CA Cancer J Clin 2022;72:7-33. [Crossref] [PubMed]
- Sung H, Ferlay J, Siegel RL, et al. Global Cancer Statistics 2020: GLOBOCAN Estimates of Incidence and Mortality Worldwide for 36 Cancers in 185 Countries. CA Cancer J Clin 2021;71:209-49. [Crossref] [PubMed]
- Tonini V, Zanni M. Pancreatic cancer in 2021: What you need to know to win. World J Gastroenterol 2021;27:5851-89. [Crossref] [PubMed]
- Hu JX, Zhao CF, Chen WB, et al. Pancreatic cancer: A review of epidemiology, trend, and risk factors. World J Gastroenterol 2021;27:4298-321. [Crossref] [PubMed]
- Khalaf N, El-Serag HB, Abrams HR, et al. Burden of Pancreatic Cancer: From Epidemiology to Practice. Clin Gastroenterol Hepatol 2021;19:876-84. [Crossref] [PubMed]
- Schizas D, Charalampakis N, Kole C, et al. Immunotherapy for pancreatic cancer: A 2020 update. Cancer Treat Rev 2020;86:102016. [Crossref] [PubMed]
- Fan JQ, Wang MF, Chen HL, et al. Current advances and outlooks in immunotherapy for pancreatic ductal adenocarcinoma. Mol Cancer 2020;19:32. [Crossref] [PubMed]
- Grover A, Sanseviero E, Timosenko E, et al. Myeloid-Derived Suppressor Cells: A Propitious Road to Clinic. Cancer Discov 2021;11:2693-706. [Crossref] [PubMed]
- Veglia F, Perego M, Gabrilovich D. Myeloid-derived suppressor cells coming of age. Nat Immunol 2018;19:108-19. [Crossref] [PubMed]
- Li K, Shi H, Zhang B, et al. Myeloid-derived suppressor cells as immunosuppressive regulators and therapeutic targets in cancer. Signal Transduct Target Ther 2021;6:362. [Crossref] [PubMed]
- Hao Z, Li R, Wang Y, et al. Landscape of Myeloid-derived Suppressor Cell in Tumor Immunotherapy. Biomark Res 2021;9:77. [Crossref] [PubMed]
- Condamine T, Gabrilovich DI. Molecular mechanisms regulating myeloid-derived suppressor cell differentiation and function. Trends Immunol 2011;32:19-25. [Crossref] [PubMed]
- Karin N. The Development and Homing of Myeloid-Derived Suppressor Cells: From a Two-Stage Model to a Multistep Narrative. Front Immunol 2020;11:557586. [Crossref] [PubMed]
- Clark CE, Hingorani SR, Mick R, et al. Dynamics of the immune reaction to pancreatic cancer from inception to invasion. Cancer Res 2007;67:9518-27. [Crossref] [PubMed]
- Bronte V, Wang M, Overwijk WW, et al. Apoptotic death of CD8+ T lymphocytes after immunization: induction of a suppressive population of Mac-1+/Gr-1+ cells. J Immunol 1998;161:5313-20. [PubMed]
- Kobayashi M, Chung JS, Beg M, et al. Blocking Monocytic Myeloid-Derived Suppressor Cell Function via Anti-DC-HIL/GPNMB Antibody Restores the In Vitro Integrity of T Cells from Cancer Patients. Clin Cancer Res 2019;25:828-38. [Crossref] [PubMed]
- Sun L, Clavijo PE, Robbins Y, et al. Inhibiting myeloid-derived suppressor cell trafficking enhances T cell immunotherapy. JCI Insight 2019;4:e126853. [Crossref] [PubMed]
- Fultang L, Panetti S, Ng M, et al. MDSC targeting with Gemtuzumab ozogamicin restores T cell immunity and immunotherapy against cancers. EBioMedicine 2019;47:235-46. [Crossref] [PubMed]
- Park JA, Wang L, Cheung NV. Modulating tumor infiltrating myeloid cells to enhance bispecific antibody-driven T cell infiltration and anti-tumor response. J Hematol Oncol 2021;14:142. [Crossref] [PubMed]
- Siret C, Collignon A, Silvy F, et al. Deciphering the Crosstalk Between Myeloid-Derived Suppressor Cells and Regulatory T Cells in Pancreatic Ductal Adenocarcinoma. Front Immunol 2020;10:3070. [Crossref] [PubMed]
- Cederbaum SD, Yu H, Grody WW, et al. Arginases I and II: do their functions overlap? Mol Genet Metab 2004;81:S38-44. [Crossref] [PubMed]
- Medina MA, Quesada AR, Núñez de Castro I, et al. Histamine, polyamines, and cancer. Biochem Pharmacol 1999;57:1341-4. [Crossref] [PubMed]
- Rodriguez PC, Quiceno DG, Ochoa AC. L-arginine availability regulates T-lymphocyte cell-cycle progression. Blood 2007;109:1568-73. [Crossref] [PubMed]
- Bogdan C. Nitric oxide and the immune response. Nat Immunol 2001;2:907-16. [Crossref] [PubMed]
- Lin CS, Ho HC, Chen KC, et al. Intracavernosal injection of vascular endothelial growth factor induces nitric oxide synthase isoforms. BJU Int 2002;89:955-60. [Crossref] [PubMed]
- Cruz MT, Duarte CB, Gonçalo M, et al. Granulocyte-macrophage colony-stimulating factor activates the transcription of nuclear factor kappa B and induces the expression of nitric oxide synthase in a skin dendritic cell line. Immunol Cell Biol 2001;79:590-6. [Crossref] [PubMed]
- Ferret-Bernard S, Saï P, Bach JM. In vitro induction of inhibitory macrophage differentiation by granulocyte-macrophage colony-stimulating factor, stem cell factor and interferon-gamma from lineage phenotypes-negative c-kit-positive murine hematopoietic progenitor cells. Immunol Lett 2004;91:221-7. [Crossref] [PubMed]
- Dawn B, Xuan YT, Guo Y, et al. IL-6 plays an obligatory role in late preconditioning via JAK-STAT signaling and upregulation of iNOS and COX-2. Cardiovasc Res 2004;64:61-71. [Crossref] [PubMed]
- Bingisser RM, Tilbrook PA, Holt PG, et al. Macrophage-derived nitric oxide regulates T cell activation via reversible disruption of the Jak3/STAT5 signaling pathway. J Immunol 1998;160:5729-34. [PubMed]
- Serafini P, Borrello I, Bronte V. Myeloid suppressor cells in cancer: recruitment, phenotype, properties, and mechanisms of immune suppression. Semin Cancer Biol 2006;16:53-65. [Crossref] [PubMed]
- Harari O, Liao JK. Inhibition of MHC II gene transcription by nitric oxide and antioxidants. Curr Pharm Des 2004;10:893-8. [Crossref] [PubMed]
- Rivoltini L, Carrabba M, Huber V, et al. Immunity to cancer: attack and escape in T lymphocyte-tumor cell interaction. Immunol Rev 2002;188:97-113. [Crossref] [PubMed]
- Sauer H, Wartenberg M, Hescheler J. Reactive oxygen species as intracellular messengers during cell growth and differentiation. Cell Physiol Biochem 2001;11:173-86. [Crossref] [PubMed]
- Kusmartsev S, Nefedova Y, Yoder D, et al. Antigen-specific inhibition of CD8+ T cell response by immature myeloid cells in cancer is mediated by reactive oxygen species. J Immunol 2004;172:989-99. [Crossref] [PubMed]
- Szuster-Ciesielska A, Hryciuk-Umer E, Stepulak A, et al. Reactive oxygen species production by blood neutrophils of patients with laryngeal carcinoma and antioxidative enzyme activity in their blood. Acta Oncol 2004;43:252-8. [Crossref] [PubMed]
- Bronte V, Kasic T, Gri G, et al. Boosting antitumor responses of T lymphocytes infiltrating human prostate cancers. J Exp Med 2005;201:1257-68. [Crossref] [PubMed]
- Nagaraj S, Gupta K, Pisarev V, et al. Altered recognition of antigen is a mechanism of CD8+ T cell tolerance in cancer. Nat Med 2007;13:828-35. [Crossref] [PubMed]
- Kusmartsev S, Nagaraj S, Gabrilovich DI. Tumor-associated CD8+ T cell tolerance induced by bone marrow-derived immature myeloid cells. J Immunol 2005;175:4583-92. [Crossref] [PubMed]
- Steinbrink K, Graulich E, Kubsch S, et al. CD4(+) and CD8(+) anergic T cells induced by interleukin-10-treated human dendritic cells display antigen-specific suppressor activity. Blood 2002;99:2468-76. [Crossref] [PubMed]
- Steinbrink K, Wölfl M, Jonuleit H, et al. Induction of tolerance by IL-10-treated dendritic cells. J Immunol 1997;159:4772-80. [PubMed]
- Porembka MR, Mitchem JB, Belt BA, et al. Pancreatic adenocarcinoma induces bone marrow mobilization of myeloid-derived suppressor cells which promote primary tumor growth. Cancer Immunol Immunother 2012;61:1373-85. [Crossref] [PubMed]
- Yan S, Wan G. Tumor-associated macrophages in immunotherapy. FEBS J 2021;288:6174-86. [Crossref] [PubMed]
- Hourani T, Holden JA, Li W, et al. Tumor Associated Macrophages: Origin, Recruitment, Phenotypic Diversity, and Targeting. Front Oncol 2021;11:788365. [Crossref] [PubMed]
- Biswas SK, Mantovani A. Macrophage plasticity and interaction with lymphocyte subsets: cancer as a paradigm. Nat Immunol 2010;11:889-96. [Crossref] [PubMed]
- Zhu S, Yi M, Wu Y, et al. Roles of tumor-associated macrophages in tumor progression: implications on therapeutic strategies. Exp Hematol Oncol 2021;10:60. [Crossref] [PubMed]
- Yang S, Liu Q, Liao Q. Tumor-Associated Macrophages in Pancreatic Ductal Adenocarcinoma: Origin, Polarization, Function, and Reprogramming. Front Cell Dev Biol 2021;8:607209. [Crossref] [PubMed]
- Zhang J, Zhou X, Hao H. Macrophage phenotype-switching in cancer. Eur J Pharmacol 2022;931:175229. [Crossref] [PubMed]
- Mantovani A, Sica A, Sozzani S, et al. The chemokine system in diverse forms of macrophage activation and polarization. Trends Immunol 2004;25:677-86. [Crossref] [PubMed]
- Martinez FO, Gordon S. The M1 and M2 paradigm of macrophage activation: time for reassessment. F1000Prime Rep 2014;6:13. [Crossref] [PubMed]
- Karp CL, Murray PJ. Non-canonical alternatives: what a macrophage is 4. J Exp Med 2012;209:427-31. [Crossref] [PubMed]
- Gordon S, Martinez FO. Alternative activation of macrophages: mechanism and functions. Immunity 2010;32:593-604. [Crossref] [PubMed]
- Habtezion A, Edderkaoui M, Pandol SJ. Macrophages and pancreatic ductal adenocarcinoma. Cancer Lett 2016;381:211-6. [Crossref] [PubMed]
- Yoshikawa K, Mitsunaga S, Kinoshita T, et al. Impact of tumor-associated macrophages on invasive ductal carcinoma of the pancreas head. Cancer Sci 2012;103:2012-20. [Crossref] [PubMed]
- von Itzstein MS, Burke MC, Brekken RA, et al. Targeting TAM to Tame Pancreatic Cancer. Target Oncol 2020;15:579-88. [Crossref] [PubMed]
- Ngambenjawong C, Gustafson HH, Pun SH. Progress in tumor-associated macrophage (TAM)-targeted therapeutics. Adv Drug Deliv Rev 2017;114:206-21. [Crossref] [PubMed]
- Burstyn-Cohen T, Maimon A. TAM receptors, Phosphatidylserine, inflammation, and Cancer. Cell Commun Signal 2019;17:156. [Crossref] [PubMed]
- Chen MM, Xiao X, Lao XM, et al. Polarization of Tissue-Resident TFH-Like Cells in Human Hepatoma Bridges Innate Monocyte Inflammation and M2b Macrophage Polarization. Cancer Discov 2016;6:1182-95. [Crossref] [PubMed]
- Bishehsari F, Zhang L, Barlass U, et al. KRAS mutation and epithelial-macrophage interplay in pancreatic neoplastic transformation. Int J Cancer 2018;143:1994-2007. [Crossref] [PubMed]
- Huang X, Li X, Ma Q, et al. Chronic alcohol exposure exacerbates inflammation and triggers pancreatic acinar-to-ductal metaplasia through PI3K/Akt/IKK. Int J Mol Med 2015;35:653-63. [Crossref] [PubMed]
- Mao X, Xu J, Wang W, et al. Crosstalk between cancer-associated fibroblasts and immune cells in the tumor microenvironment: new findings and future perspectives. Mol Cancer 2021;20:131. [Crossref] [PubMed]
- Principe DR, DeCant B, Mascariñas E, et al. TGFβ Signaling in the Pancreatic Tumor Microenvironment Promotes Fibrosis and Immune Evasion to Facilitate Tumorigenesis. Cancer Res 2016;76:2525-39. [Crossref] [PubMed]
- Daley D, Mani VR, Mohan N, et al. Dectin 1 activation on macrophages by galectin 9 promotes pancreatic carcinoma and peritumoral immune tolerance. Nat Med 2017;23:556-67. [Crossref] [PubMed]
- Eriksson E, Milenova I, Wenthe J, et al. IL-6 Signaling Blockade during CD40-Mediated Immune Activation Favors Antitumor Factors by Reducing TGF-β, Collagen Type I, and PD-L1/PD-1. J Immunol 2019;202:787-98. [Crossref] [PubMed]
- Mantovani A, Marchesi F, Malesci A, et al. Tumour-associated macrophages as treatment targets in oncology. Nat Rev Clin Oncol 2017;14:399-416. [Crossref] [PubMed]
- Lewis JS, Landers RJ, Underwood JC, et al. Expression of vascular endothelial growth factor by macrophages is up-regulated in poorly vascularized areas of breast carcinomas. J Pathol 2000;192:150-8. [Crossref] [PubMed]
- Casazza A, Laoui D, Wenes M, et al. Impeding macrophage entry into hypoxic tumor areas by Sema3A/Nrp1 signaling blockade inhibits angiogenesis and restores antitumor immunity. Cancer Cell 2013;24:695-709. [Crossref] [PubMed]
- Sakaguchi S, Mikami N, Wing JB, et al. Regulatory T Cells and Human Disease. Annu Rev Immunol 2020;38:541-66. [Crossref] [PubMed]
- Salama P, Phillips M, Grieu F, et al. Tumor-infiltrating FOXP3+ T regulatory cells show strong prognostic significance in colorectal cancer. J Clin Oncol 2009;27:186-92. [Crossref] [PubMed]
- Sugiyama D, Nishikawa H, Maeda Y, et al. Anti-CCR4 mAb selectively depletes effector-type FoxP3+CD4+ regulatory T cells, evoking antitumor immune responses in humans. Proc Natl Acad Sci U S A 2013;110:17945-50. [Crossref] [PubMed]
- Kurose K, Ohue Y, Sato E, et al. Increase in activated Treg in TIL in lung cancer and in vitro depletion of Treg by ADCC using an antihuman CCR4 mAb (KM2760). J Thorac Oncol 2015;10:74-83. [Crossref] [PubMed]
- Wang J, Gong R, Zhao C, et al. Human FOXP3 and tumour microenvironment. Immunology 2022; Epub ahead of print. [Crossref] [PubMed]
- Abbas AK, Benoist C, Bluestone JA, et al. Regulatory T cells: recommendations to simplify the nomenclature. Nat Immunol 2013;14:307-8. [Crossref] [PubMed]
- Chaudhary B, Abd Al Samid M, al-Ramadi BK, et al. Phenotypic alterations, clinical impact and therapeutic potential of regulatory T cells in cancer. Expert Opin Biol Ther 2014;14:931-45. [Crossref] [PubMed]
- Whiteside TL. Induced regulatory T cells in inhibitory microenvironments created by cancer. Expert Opin Biol Ther 2014;14:1411-25. [Crossref] [PubMed]
- Elkord E, Abd Al Samid M, Chaudhary B. Helios, and not FoxP3, is the marker of activated Tregs expressing GARP/LAP. Oncotarget 2015;6:20026-36. [Crossref] [PubMed]
- Yadav M, Stephan S, Bluestone JA. Peripherally induced tregs - role in immune homeostasis and autoimmunity. Front Immunol 2013;4:232. [Crossref] [PubMed]
- Han Y, Yang Y, Chen Z, et al. Human hepatocellular carcinoma-infiltrating CD4+CD69+Foxp3− regulatory T cell suppresses T cell response via membrane-bound TGF-β1. J Mol Med (Berl) 2014;92:539-50. [Crossref] [PubMed]
- Scurr M, Ladell K, Besneux M, et al. Highly prevalent colorectal cancer-infiltrating LAP+ Foxp3− T cells exhibit more potent immunosuppressive activity than Foxp3+ regulatory T cells. Mucosal Immunol 2014;7:428-39. [Crossref] [PubMed]
- Ghiringhelli F, Ménard C, Terme M, et al. CD4+CD25+ regulatory T cells inhibit natural killer cell functions in a transforming growth factor-beta-dependent manner. J Exp Med 2005;202:1075-85. [Crossref] [PubMed]
- Maldonado RA, von Andrian UH. How tolerogenic dendritic cells induce regulatory T cells. Adv Immunol 2010;108:111-65. [Crossref] [PubMed]
- Pedroza-Gonzalez A, Zhou G, Vargas-Mendez E, et al. Tumor-infiltrating plasmacytoid dendritic cells promote immunosuppression by Tr1 cells in human liver tumors. Oncoimmunology 2015;4:e1008355. [Crossref] [PubMed]
- Linsley PS, Bradshaw J, Greene J, et al. Intracellular trafficking of CTLA-4 and focal localization towards sites of TCR engagement. Immunity 1996;4:535-43. [Crossref] [PubMed]
- Nirschl CJ, Drake CG. Molecular pathways: coexpression of immune checkpoint molecules: signaling pathways and implications for cancer immunotherapy. Clin Cancer Res 2013;19:4917-24. [Crossref] [PubMed]
- Buchbinder EI, Desai A. CTLA-4 and PD-1 Pathways: Similarities, Differences, and Implications of Their Inhibition. Am J Clin Oncol 2016;39:98-106. [Crossref] [PubMed]
- Chambers CA, Kuhns MS, Egen JG, et al. CTLA-4-mediated inhibition in regulation of T cell responses: mechanisms and manipulation in tumor immunotherapy. Annu Rev Immunol 2001;19:565-94. [Crossref] [PubMed]
- Collins AV, Brodie DW, Gilbert RJ, et al. The interaction properties of costimulatory molecules revisited. Immunity 2002;17:201-10. [Crossref] [PubMed]
- Bennett F, Luxenberg D, Ling V, et al. Program death-1 engagement upon TCR activation has distinct effects on costimulation and cytokine-driven proliferation: attenuation of ICOS, IL-4, and IL-21, but not CD28, IL-7, and IL-15 responses. J Immunol 2003;170:711-8. [Crossref] [PubMed]
- Jie HB, Gildener-Leapman N, Li J, et al. Intratumoral regulatory T cells upregulate immunosuppressive molecules in head and neck cancer patients. Br J Cancer 2013;109:2629-35. [Crossref] [PubMed]
- Whiteside TL. What are regulatory T cells (Treg) regulating in cancer and why? Semin Cancer Biol 2012;22:327-34. [Crossref] [PubMed]
- Ladoire S, Martin F, Ghiringhelli F. Prognostic role of FOXP3+ regulatory T cells infiltrating human carcinomas: the paradox of colorectal cancer. Cancer Immunol Immunother 2011;60:909-18. [Crossref] [PubMed]
- Zhou X, Tang J, Cao H, et al. Tissue resident regulatory T cells: novel therapeutic targets for human disease. Cell Mol Immunol 2015;12:543-52. [Crossref] [PubMed]
- Weiner HL, da Cunha AP, Quintana F, et al. Oral tolerance. Immunol Rev 2011;241:241-59. [Crossref] [PubMed]
- Sakaguchi S, Yamaguchi T, Nomura T, et al. Regulatory T cells and immune tolerance. Cell 2008;133:775-87. [Crossref] [PubMed]
- Smigiel KS, Srivastava S, Stolley JM, et al. Regulatory T-cell homeostasis: steady-state maintenance and modulation during inflammation. Immunol Rev 2014;259:40-59. [Crossref] [PubMed]
- Erdman SE, Rao VP, Olipitz W, et al. Unifying roles for regulatory T cells and inflammation in cancer. Int J Cancer 2010;126:1651-65. [Crossref] [PubMed]
- Jang JE, Hajdu CH, Liot C, et al. Crosstalk between Regulatory T Cells and Tumor-Associated Dendritic Cells Negates Anti-tumor Immunity in Pancreatic Cancer. Cell Rep 2017;20:558-71. [Crossref] [PubMed]
- Daley D, Zambirinis CP, Seifert L, et al. γδ T Cells Support Pancreatic Oncogenesis by Restraining αβ T Cell Activation. Cell 2016;166:1485-1499.e15. [Crossref] [PubMed]
- Hrabák P, Kalousová M, Krechler T, et al. Pancreatic stellate cells - rising stars in pancreatic pathologies. Physiol Res 2021;70:S597-616. [Crossref] [PubMed]
- Wu Y, Zhang C, Jiang K, et al. The Role of Stellate Cells in Pancreatic Ductal Adenocarcinoma: Targeting Perspectives. Front Oncol 2021;10:621937. [Crossref] [PubMed]
- Phillips PA, McCarroll JA, Park S, et al. Rat pancreatic stellate cells secrete matrix metalloproteinases: implications for extracellular matrix turnover. Gut 2003;52:275-82. [Crossref] [PubMed]
- Krizhanovsky V, Yon M, Dickins RA, et al. Senescence of activated stellate cells limits liver fibrosis. Cell 2008;134:657-67. [Crossref] [PubMed]
- Wilson JS, Pirola RC, Apte MV. Stars and stripes in pancreatic cancer: role of stellate cells and stroma in cancer progression. Front Physiol 2014;5:52. [Crossref] [PubMed]
- Shek FW, Benyon RC, Walker FM, et al. Expression of transforming growth factor-beta 1 by pancreatic stellate cells and its implications for matrix secretion and turnover in chronic pancreatitis. Am J Pathol 2002;160:1787-98. [Crossref] [PubMed]
- Apte MV, Haber PS, Darby SJ, et al. Pancreatic stellate cells are activated by proinflammatory cytokines: implications for pancreatic fibrogenesis. Gut 1999;44:534-41. [Crossref] [PubMed]
- Hingorani SR, Petricoin EF, Maitra A, et al. Preinvasive and invasive ductal pancreatic cancer and its early detection in the mouse. Cancer Cell 2003;4:437-50. [Crossref] [PubMed]
- Mace TA, Ameen Z, Collins A, et al. Pancreatic cancer-associated stellate cells promote differentiation of myeloid-derived suppressor cells in a STAT3-dependent manner. Cancer Res 2013;73:3007-18. [Crossref] [PubMed]
- Ansari D, Ohlsson H, Althini C, et al. The Hippo Signaling Pathway in Pancreatic Cancer. Anticancer Res 2019;39:3317-21. [Crossref] [PubMed]
- Li C, Cui L, Yang L, et al. Pancreatic Stellate Cells Promote Tumor Progression by Promoting an Immunosuppressive Microenvironment in Murine Models of Pancreatic Cancer. Pancreas 2020;49:120-7. [Crossref] [PubMed]
- Liang C, Shi S, Meng Q, et al. Complex roles of the stroma in the intrinsic resistance to gemcitabine in pancreatic cancer: where we are and where we are going. Exp Mol Med 2017;49:e406. [Crossref] [PubMed]
- von Ahrens D, Bhagat TD, Nagrath D, et al. The role of stromal cancer-associated fibroblasts in pancreatic cancer. J Hematol Oncol 2017;10:76. [Crossref] [PubMed]
- Zhang YF, Jiang SH, Hu LP, et al. Targeting the tumor microenvironment for pancreatic ductal adenocarcinoma therapy. Chin Clin Oncol 2019;8:18. [Crossref] [PubMed]
- Elyada E, Bolisetty M, Laise P, et al. Cross-Species Single-Cell Analysis of Pancreatic Ductal Adenocarcinoma Reveals Antigen-Presenting Cancer-Associated Fibroblasts. Cancer Discov 2019;9:1102-23. [Crossref] [PubMed]
- Feig C, Jones JO, Kraman M, et al. Targeting CXCL12 from FAP-expressing carcinoma-associated fibroblasts synergizes with anti-PD-L1 immunotherapy in pancreatic cancer. Proc Natl Acad Sci U S A 2013;110:20212-7. [Crossref] [PubMed]
- Veenstra VL, Garcia-Garijo A, van Laarhoven HW, et al. Extracellular Influences: Molecular Subclasses and the Microenvironment in Pancreatic Cancer. Cancers (Basel) 2018.
- Sato N, Kohi S, Hirata K, et al. Role of hyaluronan in pancreatic cancer biology and therapy: Once again in the spotlight. Cancer Sci 2016;107:569-75. [Crossref] [PubMed]
- Zhou P, Li B, Liu F, et al. The epithelial to mesenchymal transition (EMT) and cancer stem cells: implication for treatment resistance in pancreatic cancer. Mol Cancer 2017;16:52. [Crossref] [PubMed]
- Huang EH, Kaufman HL. CEA-based vaccines. Expert Rev Vaccines 2002;1:49-63. [Crossref] [PubMed]
- Lei S, Appert HE, Nakata B, et al. Overexpression of HER2/neu oncogene in pancreatic cancer correlates with shortened survival. Int J Pancreatol 1995;17:15-21. [Crossref] [PubMed]
- Tang CK, Katsara M, Apostolopoulos V. Strategies used for MUC1 immunotherapy: human clinical studies. Expert Rev Vaccines 2008;7:963-75. [Crossref] [PubMed]
- Chen F, Wang W, El-Deiry WS. Current strategies to target p53 in cancer. Biochem Pharmacol 2010;80:724-30. [Crossref] [PubMed]
- Koido S, Hara E, Homma S, et al. Dendritic/pancreatic carcinoma fusions for clinical use: Comparative functional analysis of healthy- versus patient-derived fusions. Clin Immunol 2010;135:384-400. [Crossref] [PubMed]
- Koido S, Hara E, Homma S, et al. Dendritic cells fused with allogeneic colorectal cancer cell line present multiple colorectal cancer-specific antigens and induce antitumor immunity against autologous tumor cells. Clin Cancer Res 2005;11:7891-900. [Crossref] [PubMed]
- Dranoff G, Jaffee E, Lazenby A, et al. Vaccination with irradiated tumor cells engineered to secrete murine granulocyte-macrophage colony-stimulating factor stimulates potent, specific, and long-lasting anti-tumor immunity. Proc Natl Acad Sci U S A 1993;90:3539-43. [Crossref] [PubMed]
- Jaffee EM, Hruban RH, Biedrzycki B, et al. Novel allogeneic granulocyte-macrophage colony-stimulating factor-secreting tumor vaccine for pancreatic cancer: a phase I trial of safety and immune activation. J Clin Oncol 2001;19:145-56. [Crossref] [PubMed]
- Lutz E, Yeo CJ, Lillemoe KD, et al. A lethally irradiated allogeneic granulocyte-macrophage colony stimulating factor-secreting tumor vaccine for pancreatic adenocarcinoma. A Phase II trial of safety, efficacy, and immune activation. Ann Surg 2011;253:328-35. [Crossref] [PubMed]
- Wu AA, Bever KM, Ho WJ, et al. A Phase II Study of Allogeneic GM-CSF-Transfected Pancreatic Tumor Vaccine (GVAX) with Ipilimumab as Maintenance Treatment for Metastatic Pancreatic Cancer. Clin Cancer Res 2020;26:5129-39. [Crossref] [PubMed]
- Coveler AL, Rossi GR, Vahanian NN, et al. Algenpantucel-L immunotherapy in pancreatic adenocarcinoma. Immunotherapy 2016;8:117-25. [Crossref] [PubMed]
- Hardacre JM, Mulcahy M, Small W, et al. Addition of algenpantucel-L immunotherapy to standard adjuvant therapy for pancreatic cancer: a phase 2 study. J Gastrointest Surg 2013;17:94-100; discussion p. 100-1. [Crossref] [PubMed]
- Leach DR, Krummel MF, Allison JP. Enhancement of antitumor immunity by CTLA-4 blockade. Science 1996;271:1734-6. [Crossref] [PubMed]
- Hosseini A, Gharibi T, Marofi F, et al. CTLA-4: From mechanism to autoimmune therapy. Int Immunopharmacol 2020;80:106221. [Crossref] [PubMed]
- Zhang H, Dai Z, Wu W, et al. Regulatory mechanisms of immune checkpoints PD-L1 and CTLA-4 in cancer. J Exp Clin Cancer Res 2021;40:184. [Crossref] [PubMed]
- De Silva P, Aiello M, Gu-Trantien C, et al. Targeting CTLA-4 in cancer: Is it the ideal companion for PD-1 blockade immunotherapy combinations? Int J Cancer 2021;149:31-41. [Crossref] [PubMed]
- Hodi FS, O'Day SJ, McDermott DF, et al. Improved survival with ipilimumab in patients with metastatic melanoma. N Engl J Med 2010;363:711-23. [Crossref] [PubMed]
- Larkin J, Chiarion-Sileni V, Gonzalez R, et al. Five-Year Survival with Combined Nivolumab and Ipilimumab in Advanced Melanoma. N Engl J Med 2019;381:1535-46. [Crossref] [PubMed]
- Lute KD, May KF Jr, Lu P, et al. Human CTLA4 knock-in mice unravel the quantitative link between tumor immunity and autoimmunity induced by anti-CTLA-4 antibodies. Blood 2005;106:3127-33. [Crossref] [PubMed]
- Du X, Liu M, Su J, et al. Uncoupling therapeutic from immunotherapy-related adverse effects for safer and effective anti-CTLA-4 antibodies in CTLA4 humanized mice. Cell Res 2018;28:433-47. [Crossref] [PubMed]
- Du X, Tang F, Liu M, et al. A reappraisal of CTLA-4 checkpoint blockade in cancer immunotherapy. Cell Res 2018;28:416-32. [Crossref] [PubMed]
- Tawbi HA, Forsyth PA, Algazi A, et al. Combined Nivolumab and Ipilimumab in Melanoma Metastatic to the Brain. N Engl J Med 2018;379:722-30. [Crossref] [PubMed]
- Hellmann MD, Ciuleanu TE, Pluzanski A, et al. Nivolumab plus Ipilimumab in Lung Cancer with a High Tumor Mutational Burden. N Engl J Med 2018;378:2093-104. [Crossref] [PubMed]
- Motzer RJ, Tannir NM, McDermott DF, et al. Nivolumab plus Ipilimumab versus Sunitinib in Advanced Renal-Cell Carcinoma. N Engl J Med 2018;378:1277-90. [Crossref] [PubMed]
- Topalian SL, Taube JM, Anders RA, et al. Mechanism-driven biomarkers to guide immune checkpoint blockade in cancer therapy. Nat Rev Cancer 2016;16:275-87. [Crossref] [PubMed]
- Havel JJ, Chowell D, Chan TA. The evolving landscape of biomarkers for checkpoint inhibitor immunotherapy. Nat Rev Cancer 2019;19:133-50. [Crossref] [PubMed]
- Poggio M, Hu T, Pai CC, et al. Suppression of Exosomal PD-L1 Induces Systemic Anti-tumor Immunity and Memory. Cell 2019;177:414-427.e13. [Crossref] [PubMed]
- Hodi FS, Chiarion-Sileni V, Gonzalez R, et al. Nivolumab plus ipilimumab or nivolumab alone versus ipilimumab alone in advanced melanoma (CheckMate 067): 4-year outcomes of a multicentre, randomised, phase 3 trial. Lancet Oncol 2018;19:1480-92. [Crossref] [PubMed]
- Robert C, Ribas A, Schachter J, et al. Pembrolizumab versus ipilimumab in advanced melanoma (KEYNOTE-006): post-hoc 5-year results from an open-label, multicentre, randomised, controlled, phase 3 study. Lancet Oncol 2019;20:1239-51. [Crossref] [PubMed]
- Frederickson AM, Arndorfer S, Zhang I, et al. Pembrolizumab plus chemotherapy for first-line treatment of metastatic nonsquamous non-small-cell lung cancer: a network meta-analysis. Immunotherapy 2019;11:407-28. [Crossref] [PubMed]
- Atkins MB, Plimack ER, Puzanov I, et al. Axitinib in combination with pembrolizumab in patients with advanced renal cell cancer: a non-randomised, open-label, dose-finding, and dose-expansion phase 1b trial. Lancet Oncol 2018;19:405-15. [Crossref] [PubMed]
- Rini BI, Plimack ER, Stus V, et al. Pembrolizumab plus Axitinib versus Sunitinib for Advanced Renal-Cell Carcinoma. N Engl J Med 2019;380:1116-27. [Crossref] [PubMed]
- Neelapu SS, Locke FL, Bartlett NL, et al. Axicabtagene Ciloleucel CAR T-Cell Therapy in Refractory Large B-Cell Lymphoma. N Engl J Med 2017;377:2531-44. [Crossref] [PubMed]
- Winter JM, Tang LH, Klimstra DS, et al. A novel survival-based tissue microarray of pancreatic cancer validates MUC1 and mesothelin as biomarkers. PLoS One 2012;7:e40157. [Crossref] [PubMed]
- Morgan RA, Yang JC, Kitano M, et al. Case report of a serious adverse event following the administration of T cells transduced with a chimeric antigen receptor recognizing ERBB2. Mol Ther 2010;18:843-51. [Crossref] [PubMed]
- Bonifant CL, Jackson HJ, Brentjens RJ, et al. Toxicity and management in CAR T-cell therapy. Mol Ther Oncolytics 2016;3:16011. [Crossref] [PubMed]
- Chmielewski M, Hahn O, Rappl G, et al. T cells that target carcinoembryonic antigen eradicate orthotopic pancreatic carcinomas without inducing autoimmune colitis in mice. Gastroenterology 2012;143:1095-107.e2. [Crossref] [PubMed]
- Abate-Daga D, Lagisetty KH, Tran E, et al. A novel chimeric antigen receptor against prostate stem cell antigen mediates tumor destruction in a humanized mouse model of pancreatic cancer. Hum Gene Ther 2014;25:1003-12. [Crossref] [PubMed]
- Maliar A, Servais C, Waks T, et al. Redirected T cells that target pancreatic adenocarcinoma antigens eliminate tumors and metastases in mice. Gastroenterology 2012;143:1375-1384.e5. [Crossref] [PubMed]
- DeSelm CJ, Tano ZE, Varghese AM, et al. CAR T-cell therapy for pancreatic cancer. J Surg Oncol 2017;116:63-74. [Crossref] [PubMed]
- Beatty GL, Haas AR, Maus MV, et al. Mesothelin-specific chimeric antigen receptor mRNA-engineered T cells induce anti-tumor activity in solid malignancies. Cancer Immunol Res 2014;2:112-20. [Crossref] [PubMed]
- Hassan R, Laszik ZG, Lerner M, et al. Mesothelin is overexpressed in pancreaticobiliary adenocarcinomas but not in normal pancreas and chronic pancreatitis. Am J Clin Pathol 2005;124:838-45. [Crossref] [PubMed]
- Klampatsa A, Dimou V, Albelda SM. Mesothelin-targeted CAR-T cell therapy for solid tumors. Expert Opin Biol Ther 2021;21:473-86. [Crossref] [PubMed]
- Morello A, Sadelain M, Adusumilli PS. Mesothelin-Targeted CARs: Driving T Cells to Solid Tumors. Cancer Discov 2016;6:133-46. [Crossref] [PubMed]
- Faust JR, Hamill D, Kolb EA, et al. Mesothelin: An Immunotherapeutic Target beyond Solid Tumors. Cancers (Basel) 2022.
- Thomas AM, Santarsiero LM, Lutz ER, et al. Mesothelin-specific CD8(+) T cell responses provide evidence of in vivo cross-priming by antigen-presenting cells in vaccinated pancreatic cancer patients. J Exp Med 2004;200:297-306. [Crossref] [PubMed]
- Montemagno C, Cassim S, Pouyssegur J, et al. From Malignant Progression to Therapeutic Targeting: Current Insights of Mesothelin in Pancreatic Ductal Adenocarcinoma. Int J Mol Sci 2020; [Crossref] [PubMed]
- Zheng C, Jia W, Tang Y, et al. Mesothelin regulates growth and apoptosis in pancreatic cancer cells through p53-dependent and -independent signal pathway. J Exp Clin Cancer Res 2012;31:84. [Crossref] [PubMed]
- Rump A, Morikawa Y, Tanaka M, et al. Binding of ovarian cancer antigen CA125/MUC16 to mesothelin mediates cell adhesion. J Biol Chem 2004;279:9190-8. [Crossref] [PubMed]
- Beatty GL, O'Hara MH, Lacey SF, et al. Activity of Mesothelin-Specific Chimeric Antigen Receptor T Cells Against Pancreatic Carcinoma Metastases in a Phase 1 Trial. Gastroenterology 2018;155:29-32. [Crossref] [PubMed]
- Luheshi NM, Coates-Ulrichsen J, Harper J, et al. Transformation of the tumour microenvironment by a CD40 agonist antibody correlates with improved responses to PD-L1 blockade in a mouse orthotopic pancreatic tumour model. Oncotarget 2016;7:18508-20. [Crossref] [PubMed]
- Sanford DE, Belt BA, Panni RZ, et al. Inflammatory monocyte mobilization decreases patient survival in pancreatic cancer: a role for targeting the CCL2/CCR2 axis. Clin Cancer Res 2013;19:3404-15. [Crossref] [PubMed]
- Nywening TM, Wang-Gillam A, Sanford DE, et al. Targeting tumour-associated macrophages with CCR2 inhibition in combination with FOLFIRINOX in patients with borderline resectable and locally advanced pancreatic cancer: a single-centre, open-label, dose-finding, non-randomised, phase 1b trial. Lancet Oncol 2016;17:651-62. [Crossref] [PubMed]
- Zhu Y, Knolhoff BL, Meyer MA, et al. CSF1/CSF1R blockade reprograms tumor-infiltrating macrophages and improves response to T-cell checkpoint immunotherapy in pancreatic cancer models. Cancer Res 2014;74:5057-69. [Crossref] [PubMed]
- Wiehagen KR, Girgis NM, Yamada DH, et al. Combination of CD40 Agonism and CSF-1R Blockade Reconditions Tumor-Associated Macrophages and Drives Potent Antitumor Immunity. Cancer Immunol Res 2017;5:1109-21. [Crossref] [PubMed]
- Steele CW, Karim SA, Leach JDG, et al. CXCR2 Inhibition Profoundly Suppresses Metastases and Augments Immunotherapy in Pancreatic Ductal Adenocarcinoma. Cancer Cell 2016;29:832-45. [Crossref] [PubMed]
- Kumar V, Donthireddy L, Marvel D, et al. Cancer-Associated Fibroblasts Neutralize the Anti-tumor Effect of CSF1 Receptor Blockade by Inducing PMN-MDSC Infiltration of Tumors. Cancer Cell 2017;32:654-668.e5. [Crossref] [PubMed]
- Bullock K, Richmond A. Suppressing MDSC Recruitment to the Tumor Microenvironment by Antagonizing CXCR2 to Enhance the Efficacy of Immunotherapy. Cancers (Basel) 2021.
- Manuel ER, Chen J, D'Apuzzo M, et al. Salmonella-Based Therapy Targeting Indoleamine 2,3-Dioxygenase Coupled with Enzymatic Depletion of Tumor Hyaluronan Induces Complete Regression of Aggressive Pancreatic Tumors. Cancer Immunol Res 2015;3:1096-107. [Crossref] [PubMed]
- Erkan M, Hausmann S, Michalski CW, et al. The role of stroma in pancreatic cancer: diagnostic and therapeutic implications. Nat Rev Gastroenterol Hepatol 2012;9:454-67. [Crossref] [PubMed]
- Yuan C, Qian ZR, Babic A, et al. Prediagnostic Plasma 25-Hydroxyvitamin D and Pancreatic Cancer Survival. J Clin Oncol 2016;34:2899-905. [Crossref] [PubMed]
- Sherman MH, Yu RT, Engle DD, et al. Vitamin D receptor-mediated stromal reprogramming suppresses pancreatitis and enhances pancreatic cancer therapy. Cell 2014;159:80-93. [Crossref] [PubMed]
- Lo A, Li CP, Buza EL, et al. Fibroblast activation protein augments progression and metastasis of pancreatic ductal adenocarcinoma. JCI Insight 2017;2:92232. [Crossref] [PubMed]
- Lo A, Wang LS, Scholler J, et al. Tumor-Promoting Desmoplasia Is Disrupted by Depleting FAP-Expressing Stromal Cells. Cancer Res 2015;75:2800-10. [Crossref] [PubMed]
- Xiong HQ, Rosenberg A, LoBuglio A, et al. Cetuximab, a monoclonal antibody targeting the epidermal growth factor receptor, in combination with gemcitabine for advanced pancreatic cancer: a multicenter phase II Trial. J Clin Oncol 2004;22:2610-6. [Crossref] [PubMed]
- Tai CJ, Huang MT, Wu CH, et al. Combination of Two Targeted Medications (Bevacizumab Plus Cetuximab) Improve the Therapeutic Response of Pancreatic Carcinoma. Medicine (Baltimore) 2016;95:e3259. [Crossref] [PubMed]
- Faloppi L, Andrikou K, Cascinu S. Cetuximab: still an option in the treatment of pancreatic cancer? Expert Opin Biol Ther 2013;13:791-801. [Crossref] [PubMed]
- Bischoff JR, Kirn DH, Williams A, et al. An adenovirus mutant that replicates selectively in p53-deficient human tumor cells. Science 1996;274:373-6. [Crossref] [PubMed]
- Hecht JR, Bedford R, Abbruzzese JL, et al. A phase I/II trial of intratumoral endoscopic ultrasound injection of ONYX-015 with intravenous gemcitabine in unresectable pancreatic carcinoma. Clin Cancer Res 2003;9:555-61. [PubMed]
- Oberg D, Yanover E, Adam V, et al. Improved potency and selectivity of an oncolytic E1ACR2 and E1B19K deleted adenoviral mutant in prostate and pancreatic cancers. Clin Cancer Res 2010;16:541-53. [Crossref] [PubMed]
- Fu X, Tao L, Li M, et al. Effective treatment of pancreatic cancer xenografts with a conditionally replicating virus derived from type 2 herpes simplex virus. Clin Cancer Res 2006;12:3152-7. [Crossref] [PubMed]
- Kasuya H, Nishiyama Y, Nomoto S, et al. Suitability of a US3-inactivated HSV mutant (L1BR1) as an oncolytic virus for pancreatic cancer therapy. Cancer Gene Ther 2007;14:533-42. [Crossref] [PubMed]
- Penheiter AR, Wegman TR, Classic KL, et al. Sodium iodide symporter (NIS)-mediated radiovirotherapy for pancreatic cancer. AJR Am J Roentgenol 2010;195:341-9. [Crossref] [PubMed]
- Bossow S, Grossardt C, Temme A, et al. Armed and targeted measles virus for chemovirotherapy of pancreatic cancer. Cancer Gene Ther 2011;18:598-608. [Crossref] [PubMed]
- Wirth T, Zender L, Schulte B, et al. A telomerase-dependent conditionally replicating adenovirus for selective treatment of cancer. Cancer Res 2003;63:3181-8. [PubMed]
- Eriksson E, Moreno R, Milenova I, et al. Activation of myeloid and endothelial cells by CD40L gene therapy supports T-cell expansion and migration into the tumor microenvironment. Gene Ther 2017;24:92-103. [Crossref] [PubMed]
- Rodríguez-García A, Giménez-Alejandre M, Rojas JJ, et al. Safety and efficacy of VCN-01, an oncolytic adenovirus combining fiber HSG-binding domain replacement with RGD and hyaluronidase expression. Clin Cancer Res 2015;21:1406-18. [Crossref] [PubMed]
- Guedan S, Rojas JJ, Gros A, et al. Hyaluronidase expression by an oncolytic adenovirus enhances its intratumoral spread and suppresses tumor growth. Mol Ther 2010;18:1275-83. [Crossref] [PubMed]
- Rahal A, Musher B. Oncolytic viral therapy for pancreatic cancer. J Surg Oncol 2017;116:94-103. [Crossref] [PubMed]
- Yu YA, Galanis C, Woo Y, et al. Regression of human pancreatic tumor xenografts in mice after a single systemic injection of recombinant vaccinia virus GLV-1h68. Mol Cancer Ther 2009;8:141-51. [Crossref] [PubMed]
- Tysome JR, Briat A, Alusi G, et al. Lister strain of vaccinia virus armed with endostatin-angiostatin fusion gene as a novel therapeutic agent for human pancreatic cancer. Gene Ther 2009;16:1223-33. [Crossref] [PubMed]
- Wennier ST, Liu J, Li S, et al. Myxoma virus sensitizes cancer cells to gemcitabine and is an effective oncolytic virotherapeutic in models of disseminated pancreatic cancer. Mol Ther 2012;20:759-68. [Crossref] [PubMed]
- Woo Y, Kelly KJ, Stanford MM, et al. Myxoma virus is oncolytic for human pancreatic adenocarcinoma cells. Ann Surg Oncol 2008;15:2329-35. [Crossref] [PubMed]
Cite this article as: Yu X, Shi S, Yu X. Immune microenvironment and immunotherapy in pancreatic cancer: a narrative review. Precis Cancer Med 2022;5:39.